Albert Einstein publishes his theory of Special Relativity
On 30 June 1905 the German physics journal…
Know moreErwin Schrödinger and Werner Heisenberg devise a quantum theory
In the 1920s, physicists were trying to apply…
Know moreThe Bevatron starts up at Berkeley, California
The Bevatron in 1958 (Image: Lawrence Berkeley…
Know moreCronin and Fitch detect a difference between matter and antimatter
In 1964, James Cronin and Val Fitch at Brookhaven…
Know moreFirst antiatoms produced: antihydrogen, at CERN
A team led by Walter Oelert created atoms of…
Know moreATHENA and ATRAP create "cold" antimatter
Two CERN experiments, ATHENA and ATRAP, created…
Know moreALPHA traps antimatter atoms for 1000 seconds
The ALPHA experiment at CERN reported today that…
Know moreASACUSA weighs antimatter to one part in a billion
In a paper published today in the journal Nature…
Know moreCERN sets course for extra-low-energy antiprotons
The kick-off meeting for ELENA, the Extra Low…
Know moreATRAP experiment makes world’s most precise measurement of antiproton magnetic moment
In Physical Review Letters, the Antihydrogen…
Know moreLHCb experiment observes new matter-antimatter difference
The LHCb collaboration at CERN publishes a…
Know moreALPHA first direct analysis on how antimatter is affected by gravity
The ALPHA collaboration at CERN1 has…
Know moreCERN’s ALPHA experiment measures charge of antihydrogen
In a paper published in the journal Nature…
Know moreA cut-away schematic of the Penning trap system used by BASE. The experiment receives antiprotons from CERN's AD; negative hydrogen ions are formed during injection into the apparatus. (Image: CERN) The BASE experiment at CERN compares protons and antiprotons with high precision
Geneva, 12 August 2015. In a paper published…
Know moreASACUSA experiment improves precision of antiproton mass measurement with new innovative cooling…
The ASACUSA experiment at CERN reports in Science…
Know moreALPHA experiment observes the light spectrum of antimatter for the first time
The ALPHA collaboration reports in Nature the…
Know moreThe BASE experiment breaks its own precision measurement record of antiproton’s magnetic moment
The BASE collaboration, publishes today in …
Know moreA CP-symmetry transformation swaps a particle with the mirror image of its antiparticle. (Image: CERN) LHCb sees a new flavour of matter–antimatter asymmetry
The LHCb collaboration at CERN has seen, for the…
Know moreALPHA cools antimatter using laser light for the first time
The ALPHA collaboration at CERN has succeeded in…
Know more
Embed this timeline
Albert Einstein publishes his theory of Special Relativity
Victor Hess discovers cosmic rays
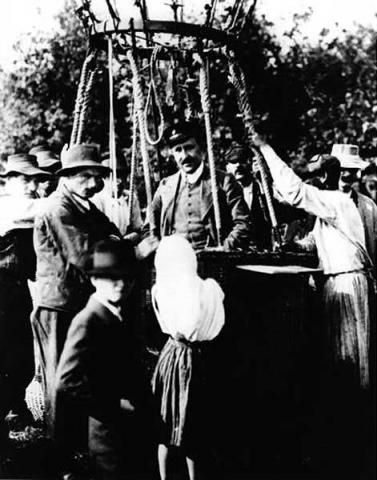
Erwin Schrödinger and Werner Heisenberg devise a quantum theory
Dirac's equation predicts antiparticles
Carl Anderson discovers the positron
Ernest Lawrence patents the cyclotron
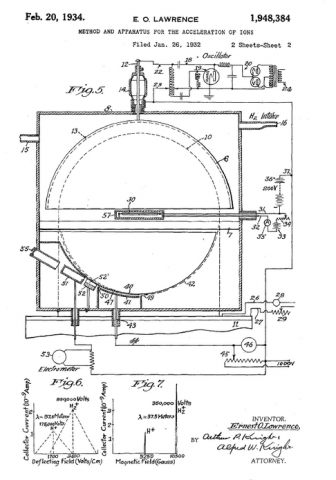
The Bevatron starts up at Berkeley, California
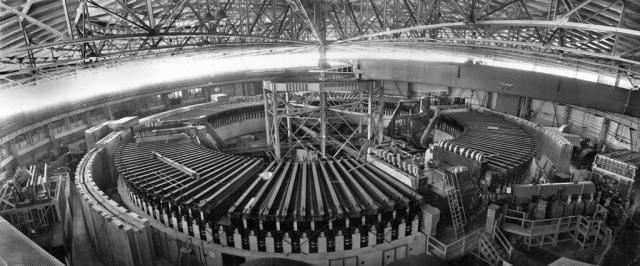
The Bevatron discovers the antiproton
The Bevatron discovers the antineutron
Cronin and Fitch detect a difference between matter and antimatter
First observations of antinuclei
First storage of antiprotons
First proton-antiproton collisions
First antiatoms produced: antihydrogen, at CERN
Antiproton Decelerator approved
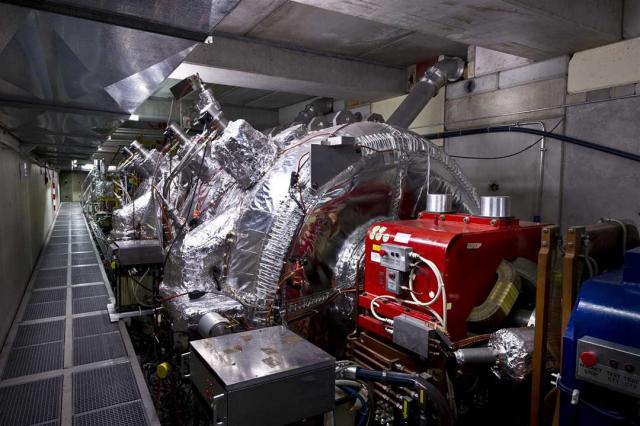